Autores
Custodio, M.F. (UNICAMP) ; de Araujo, W.R. (UNICAMP)
Resumo
In this study, we developed an electroanalytical device using the laser-scribed
graphene (LSG) method onto polyetherimide substrate for the detection of uric
acid (UA) in saliva samples. The electrode underwent an anodic pretreatment (AT)
and was later modified with gold nanoparticles (AuNPs). The AuNPs/AT/LSG sensor
exhibited high sensitivity and selectivity performance for UA detection in
phosphate buffer solution (pH = 7.0). The limit of detection was 1.34 μM. We
demonstrate that UA can be detected without significant interference from some
biomarkers that are normally present in saliva. The sensor was successfully
applied in the analysis of UA in artificial saliva samples and the results
indicated that the developed device is promising for the non-invasive monitoring
of salivary UA.
Palavras chaves
Electrochemical sensor; Uric acid; Diagnostics
Introdução
Saliva is an excellent diagnostic fluid that provides an alternative to direct
blood analysis through the permeation of blood constituents without any skin
perforation for collection (KIM et al., 2015). The composition of saliva can
reflect an individual's hormonal, emotional, metabolic, nutritional, and
immunological status (GLEERUP et al., 2021). Saliva testing is currently used in
the fields of toxicology, endocrinology, infectious diseases, and forensics. As
saliva collection is non-invasive and relatively stress-free, saliva may serve
as a potential alternative and universal diagnostic fluid (CARRO et al., 2017).
Uric Acid (UA) is one of the main biomolecules present in physiological liquids
and plays a crucial role in human metabolic processes (ELANGOVAN et al., 2020,
SAMOSON et al., 2022). It is the final product of purine metabolism (GHOREISHI;
BEHPOUR; SAEIDINEJAD, 2012). Monitoring the uric acid levels in the body fluids
is of great importance, as abnormal levels of UA in human biofluids serve as an
essential marker (XU et al., 2015, SAMOSON et al., 2022) of various diseases
such as gout, hyperuricemia, kidney disease, Lesch-Nyhan syndrome, hypertension,
and high cholesterol (GHOREISHI; BEHPOUR; SAEIDINEJAD, 2012, ELANGOVAN et al.,
2020).
The most used methods to determine UA include fluorescence spectroscopy,
capillary electrophoresis, high-performance liquid chromatography, and bio-
enzymatic colorimetry. While most of these methods are accurate and sensitive,
operating costs can be high and the techniques used are unsuitable for analysis
at the point of need (SAMOSON et al., 2022). Therefore, developing sensitive,
simple, and fast analytical methods for detecting UA in routine analysis is
essential (GHOREISHI; BEHPOUR; SAEIDINEJAD, 2012; GUAN et al., 2021).
A promising alternative approach to UA detection is the use of electrochemical
sensors, which is generally simple, inexpensive, fast, highly sensitive, and
suitable for on-site monitoring. UA can be detected through non-enzymatic and
enzymatic analyses (XU et al., 2015). Recently developed non-enzymatic UA
sensors have employed various transition metal nanoparticles (MNPs) as catalytic
materials, where UA can be detected through its oxidation at an electrode
modified with a more stable catalytic material (SAMOSON et al., 2022).
In the last decades, electrodes modified with carbonaceous material have been
extensively researched in the field of electroanalytical chemistry. Among them,
graphene (XU et al., 2018) has gained enormous attraction by the scientific
community due to its unique properties such as rapid electronic mobility, self-
assembly behavior, excellent conductivity, mechanical stability and flexibility
(LAHCEN et al., 2020). In addition, graphene-based materials provide improved
electrochemical performance in biochemical sensing (XU et al., 2018).
Laser scribing of different substrates has emerged (RAUF et al., 2021) as a new
method for 3D graphene production and has advantages over the chemical vapor
deposition (CVD) process (LAHCEN et al., 2020). This new technique has only one
step and its product is called laser scribed graphene (LSG). It is a method that
allows the modification of a substrate into a porous 3D graphene structure,
exhibiting high electrical conductivity (CARDOSO et al., 2019). The nature of
chemical repeating units present in the substrate plays a key role in the
fabrication of LSG electrodes (LAHCEN et al., 2020). For example, a brief
literature review showed that polyimide (PI) and PEI substrates are successfully
converted to graphene, while polymers with non-aromatic groups undergo almost
complete degradation without any graphene formation (LAHCEN et al., 2020).
Chemical modification of the electrode surface is a strategy to improve the
performance of conventional electrodes for various applications, especially
sensors (GHOREISHI; BEHPOUR; SAEIDINEJAD, 2012). Gold nanoparticles (AuNPs) have
shown excellent performance in sensing, electronics, catalyst, and biomedical
applications (ELANGOVAN et al., 2020) due to their special optical and
electronic properties (GUAN et al., 2021). Due to their small dimensional size,
good conductivity, and excellent catalytic activity, AuNPs have potential
applications in the preparation of electrochemical sensors and biosensors where
they work by efficiently channeling electrons between the electrode surface and
the electrolyte (GHOREISHI; BEHPOUR; SAEIDINEJAD, 2012). In addition, AuNPs when
integrated with carbon-based nanomaterials increase the electrocatalytic
performance and sensitivity of the sensors (SAMOSON et al., 2022).
In this work, we present the development of a portable electrochemical sensor
using the LSG method on PEI polymer. The working electrode was modified with
AuNPs by drop-casting technique for sensitive and non-enzymatic detection of
uric acid present in saliva samples.
Material e métodos
All reagents used were of analytical grade. AuNPs suspension was synthesized by
the hydrothermal method according to Melo Jr. and collaborators (MELO JR. et
al., 2012). Synthetic saliva was prepared from a mixture of 13.2 mg of NaCl,
96.4 mg of KCl, 17.2 mg of CaCl2, 65.0 mg of KH2PO4, 20.4 mg of urea, and 17.4
mg of lactic acid in 100 ml of deionized water.
The LSG electrochemical sensors were fabricated onto PEI substrate using a CO2
laser printer equipped with a 50W laser tube (Multivisi – Visutec Router
VS4040C, Brazil). For the fabrication of the electrode design, the Corel Draw®
was used, the laser power was optimized as 11%, and the scan rate was 40 mm s-1.
Then, Ag/AgCl conductive ink from Creative Materials® was painted to create the
pseudoreference electrode. The ink was cured at 100 ºC for 30 min in a drying
oven FANEM® SP-Brasil model 315 SE. Finally, Norbond® Saint Gobain insulating
tape was applied to delimit the electrode area and the electrochemical cell.
Electrochemical measurements were performed using a PalmSens3
potentiostat/galvanostat (Randhoeve, Houten, Netherlands) with a laptop running
the PSTrace 5.9 software. First, to evaluate the sensor response, measurements
were performed with and without electrochemical pretreatments. The anodic
treatment (AT) consists of applying the potential of +1.8 V for 200 s in 0.1 mol
L-1 NaOH, and the cathodic treatment (CT) consisted in applying the potential of
-1.5 V for 200 s in 0.1 mol L-1 H2SO4. The voltammetric characterization of the
electrodes and UA was performed by cyclic voltammetry (CV) technique using 100
µL of 0.1 mol L-1 phosphate buffer solution (PBS), pH 7.4, in the potential
range of -0.2 to 1.0 V at 50 mV s-1. For the mass transport study, CV
measurements were performed in the scan rate values ranging from 10 to 200 mV s-
1 using 0.1 mol L-1 PBS pH 7.4 as supporting electrolyte and 0.5 mmol L-1 UA
solution. To study the effect of pH on the voltammetric response of UA, analyses
were performed with pH values in the range of 5 to 9.
The analytical curve was built using differential pulse voltammetry (DPV) in the
potential range of -0.4 to +0.5 V at a scan rate of 0.03 V s-1, with the step of
5 mV and amplitude of 50 mV. Measurements were performed in triplicate using
different sensors.
To evaluate the accuracy of the proposed method, an addition and recovery study
was carried out in which concentrations of 200 and 400 µmol L-1 were spiked into
prepared saliva samples to mimic the usual levels for healthy and hyperuricemic
individuals, respectively. For analysis of spiked samples, a 20-fold dilution of
synthetic saliva in PBS pH=7.0 was performed. Solutions of lactic acid, glucose,
creatinine, and ascorbic acid were prepared and analyzed to evaluate the
selectivity of the method. These tests were carried out under optimized
conditions using a 1:1 proportion between uric acid:interfering species at 100
μmol L-1.
Resultado e discussão
Cyclic voltammograms were recorded in the potential range of -0.2 to 1.0 V at 50
mV s-1 in PBS medium to evaluate the response of UA in our LSG sensor with and
without electrochemical treatments, Figure 1A. It is possible to verify that the
LSG electrode presented a low and stable background signal (blank solution),
which is very attractive for use as an electrochemical sensor. When UA was
added, it showed a poorly defined irreversible oxidation process under the
conditions used. Subsequently, some electrochemical treatments were evaluated,
aiming to introduce functional groups on the LSG surface that can act as
catalytic centers for electronic transfer. Figure 1A exhibited the comparison of
the electrochemical performance of the LSG sensor before and after AT and CT
treatments. It is noteworthy that both surface treatments of the electrode
caused a significant increase in current signals with superior kinetics and a
catalytic effect. Given the best analytical response was obtained for the AT
condition in NaOH 0.1 mol L-1, this condition was chosen to further studies.
After choosing the treatment, the drop-casting modification of the sensor with
AuNPs was studied by varying the volume applied to the electrode surface from 1
to 5 µL, Figure 1B. The AuNPs were used aiming to improve the analytical
performance of electrodes, both by increasing surface area and the possibility
of associating catalytic effects with its use. After adding the AuNPs, it was
allowed to dry for 30 min. The blank and analyte were measured by CV for each
modified electrode in the potential range of -0.4 to 0.5 V. It was observed that
with the increase in the amount of AuNPs used in the surface modification of the
working electrode, there was an increase in the peak current of the UA. The best
analytical condition was the use of 5 µL of AuNPs since it provided the highest
and a well-defined peak current, Figure 1B.
Subsequently, a pH study was carried out in the pH range of 5 to 9, Figure 1C.
The resulting plot of the peak potential of UA oxidation versus pH is shown in
Figure 1D. The highest oxidation current intensity was obtained in a more acidic
medium and there is a tendency to decrease with increasing pH. The peak
potential values decreased linearly with increasing pH at a rate of 83 mV/pH,
suggesting that the oxidation process occurs with the same proportion of
electrons and protons. Considering that the objective is to work in conditions
closest to the reality of the salivary environment, we can define pH = 7.0 as
the best way to proceed since it is the pH corresponding to the most common
salivary pH range (6.8 - 7.2), according to the existing consensus in the
literature on oral pH (TOLENTINO, 2009). In addition, pH = 7.0 provided
anticipation in the detection potential compared to acidic medium (close to 0V),
which minimize selectivity issues.
To investigate the mass transport phenomena involved in the redox processes of
uric acid, the scan rate study was performed, Figure 1E. In this study, the uric
acid anodic process has a slope of 0.824, Figure 1F, indicating an adsorptive
characteristic of the electrochemical process.
Figure 1: (A) CVs obtained for 0.5 mmol L-1 UA without treatment (black line),
with anodic treatment (red line), and with cathodic treatment (blue line) of the
LSG sensor at 50 mV s-1. Inserted in A is the expanded CV recorded for PBS pH
7.4 (dashed line) and 0.5 mmol L-1 UA (black line) using LSG without any
previous treatment. (B) CVs recorded for the optimization study of AuNPs used to
modify the treated sensor. 0.5 mmol L-1 UA was used without AuNPs (black line),
with 1 µL of AuNPs (green line), 3 µL of AuNPs (blue line), and 5 µL of AuNPs
(red line). (C) Study of the effect of pH on the 0.5 mmol L-1 UA response in the
sensor with optimized conditions in the pH range of 5.0 (black line) to 9.0
(magenta line). (D) Graph of peak potential versus pH for UA response. (E) CVs
were recorded using the LSG sensor with optimized conditions containing 0.5 mmol
L−1 UA in PBS medium, pH = 7.0, at different scan rates (10, 20, 30, 40, 100,
and 200 mV s-1). (F) The plot of the logarithm of peak current versus the
logarithm of scan rate (v).
After the optimization of the AuNPs/AT/LSG sensor (laser-scribed
graphene/anodically treated/modified with gold nanoparticles), a DPV technique
was used and the main instrumental parameters were optimized to improve the
sensitivity of the method. A 0.1 mmol L-1 UA response was evaluated by changing
the step and amplitude potential from 3 to 12 mV and from 30 to 120 mV,
respectively. The best conditions were obtained when using a step of 5 mV and an
amplitude of 50 mV.
The analytical curve was obtained in the concentration ranging from 2 to 200
μmol L−1 UA using optimized parameters. Figures 2A and 2B show the DPV plots and
respective analytical curve using the peak current from the DPV data. We
identified two linear regions, the first from 2 to 60 μmol L−1 UA and the second
from 60 to 200 μmol L−1 UA. For the first linear region, we have a sensitivity
of 0.094 μA/μmol L−1 and an R2 of 0.998. For the second linear region, the
sensitivity is 0.046 μA/μmol L−1, and the R2 of 0.971. The limit of detection
(LOD) and the limit of quantification (LOQ) were calculated as 1.34 μmol L−1 and
4.46 μmol L−1, respectively.
Figure 2: (A) DPV recorded with AuNPs/AT/LSG electrode in PBS medium, pH 7.0, in
the concentration ranging from 2 to 200 μmol L−1 UA. (B) Analytical curve
constructed using the average of the peak currents including the error bars (n =
3). Parameters: potential from -0.4 to +0.5 V; scan rate of 0.03 V s-1; step of
5 mV and amplitude of 50 mV.
Considering that the salivary environment is composed of a multitude of
biomarkers, the potential interference of the major compounds found in biofluids
were evaluated, i.e., ascorbic acid (AA), lactic acid (Lac), glucose (Glu), and
creatinine (Cre). When evaluating the UA response in the presence of these
interfering species, it was found that Glu caused a 9.3% peak current variation,
and all other species provided relative current variations lower than 3.7%,
highlighting the adequate selectivity of the non-enzymatic LSG sensor.
To assess the applicability of the proposed sensor (AuNPs/AT/LSG) in practical
analysis, the electrochemical response was studied in artificial saliva that has
an electrolyte concentration similar to human saliva. Saliva was prepared
according to the method used by Bao et al. (BAO; KAUR; KIM, 2019) and its pH was
adjusted to 6.9. Before electrochemical detection of UA, saliva samples
containing 200 and 400 µmol L-1 UA were prepared and then diluted 20x in PBS
solution, pH 7.0. The dilution process was necessary to work within the linear
range of our method, considering that healthy patients have UA concentrations
around 200 µmol L-1 and clinical patients who suffer from gout, for example,
have much higher levels of UA concentrations (~400 µmol L-1). In this way, a
standard addition method was performed for salivary UA quantification in these
samples, simulating the application of the proposed sensor for real cases. Table
1 shows the results obtained, with recoveries ranging from 94 to 120%, which
confirms the accuracy of the AuNPs/AT/LSG sensor for the determination of
salivary UA.
Table 1: Detection of uric acid in artificial saliva samples using the
AuNPs/AT/LSG sensor.
The concentration of added uric acid (μmol L-1) Uric acid detected (μmol L-1)
Recovery (%)
200.0 240.0 120.0%
200.0 188.2 94.1%
400.0 436.0 109.0%
400.0 390.0 97.5%
This work presented a non-enzymatic sensor for salivary uric acid detection with
a low LOD and wide working concentration range. The proposed LSG/AT/AuNPs
electrode presents analytical performance comparable or superior to those
obtained with several other sensors, including enzymatic ones.
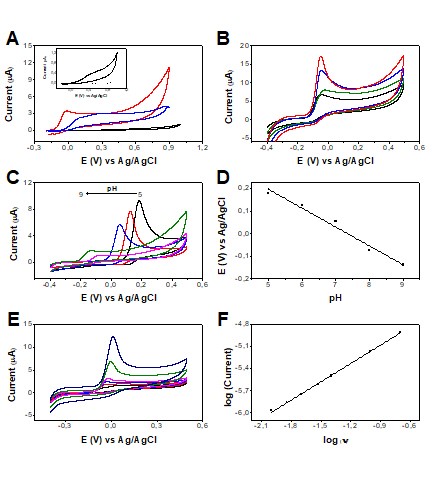
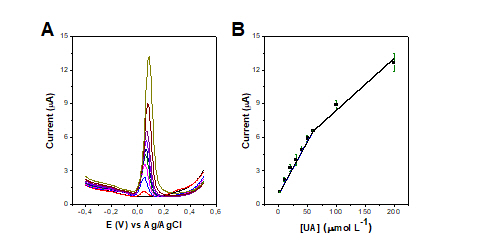
Conclusões
This work described a portable, accessible, and non-enzymatic electrochemical
sensor for UA detection using a CO2 laser-scribing procedure on PEI substrate. The
LSG electrodes were anodically treated in 0.1 mol L-1 NaOH and modified with AuNP
suspension to improve the analytical and catalytical performance of the
electrodes. The AuNPs/AT/LSG electrode features high sensitivity of 0.094 μA/μmol
L−1, a working concentration range of 2 μM – 200 μM, and a low LOD of 1.34 μM. The
selectivity of the method was studied by evaluating the major salivary biomarkers
such as glucose, lactate, creatinine, and ascorbic acid. They did not show
significant changes (< 9.3%) in the UA response, confirming adequate selectivity
of our method. The recovery studies performed in artificial saliva samples spiked
with UA similar to healthy and hyperuricemic conditions resulted in recoveries in
the range of 94 to 120%, demonstrating the accuracy and robustness of the
AuNPs/AT/LSG sensor toward noninvasive monitoring of UA in saliva samples.
Agradecimentos
The authors acknowledge the IQ-UNICAMP and the Brazilian agencies CAPES, CNPq, and
FAPESP. A special thanks to FAEPEX/PRP/UNICAMP for the master's scholarship
(Process: 2387/21).
Referências
BAO, C.; KAUR, M.; KIM, W. S. Toward a highly selective artificial saliva sensor using printed hybrid field effect transistors. Sensors and Actuators B: Chemical, v. 285, p. 186–192, abr. 2019. Disponível em: <https://linkinghub.elsevier.com/retrieve/pii/S0925400519300796>.
CARDOSO, A. R.; MARQUES, A. C.; SANTOS, L.; CARVALHO, A. F.; COSTA, F. M.; MARTINS, R.; SALES, M. G. F.; FORTUNATO, E. Molecularly-imprinted chloramphenicol sensor with laser-induced graphene electrodes. Biosensors and Bioelectronics, v. 124–125, p. 167–175, jan. 2019. Disponível em: <https://linkinghub.elsevier.com/retrieve/pii/S0956566318308285>.
CARRO, E.; BARTOLOMÉ, F.; BERMEJO‐PAREJA, F.; VILLAREJO‐GALENDE, A.; MOLINA, J. A.; ORTIZ, P.; CALERO, M.; RABANO, A.; CANTERO, J. L.; ORIVE, G. Early diagnosis of mild cognitive impairment and Alzheimer’s disease based on salivary lactoferrin. Alzheimer’s & Dementia: Diagnosis, Assessment & Disease Monitoring, v. 8, n. 1, p. 131–138, 26 jan. 2017. Disponível em: <https://onlinelibrary.wiley.com/doi/abs/10.1016/j.dadm.2017.04.002>.
ELANGOVAN, A.; SUDHA, K.; JEEVIKA, A.; BHUVANESHWARI, C.; KALIMUTHU, P.; BALAKUMAR, V. Construction of ternary Au@GO coupled with poly-l-ethionine nanocomposite as a robust platform for electrochemical recognition of uric acid in diabetic patients. Colloids and Surfaces A: Physicochemical and Engineering Aspects, v. 602, p. 125050, out. 2020. Disponível em: <https://linkinghub.elsevier.com/retrieve/pii/S0927775720306439>.
GHOREISHI, S. M.; BEHPOUR, M.; SAEIDINEJAD, F. Electrochemical determination of tryptophan, uric acid and ascorbic acid at a gold nanoparticles modified carbon paste electrode. Analytical Methods, v. 4, n. 8, p. 2447, 2012. Disponível em: <http://xlink.rsc.org/?DOI=c2ay00017b>.
GLEERUP, H. S.; JENSEN, C. S.; HØGH, P.; HASSELBALCH, S. G.; SIMONSEN, A. H. Lactoferrin in cerebrospinal fluid and saliva is not a diagnostic biomarker for Alzheimer’s disease in a mixed memory clinic population. EBioMedicine, v. 67, p. 103361, maio 2021. Disponível em: <https://linkinghub.elsevier.com/retrieve/pii/S2352396421001547>.
GUAN, Q.; GUO, H.; XUE, R.; WANG, M.; ZHAO, X.; FAN, T.; YANG, W.; XU, M.; YANG, W. Electrochemical sensor based on covalent organic frameworks-MWCNT-NH2/AuNPs for simultaneous detection of dopamine and uric acid. Journal of Electroanalytical Chemistry, v. 880, p. 114932, jan. 2021. Disponível em: <https://linkinghub.elsevier.com/retrieve/pii/S1572665720311619>.
KIM, J.; IMANI, S.; DE ARAUJO, W. R.; WARCHALL, J.; VALDÉS-RAMÍREZ, G.; PAIXÃO, T. R. L. C.; MERCIER, P. P.; WANG, J. Wearable salivary uric acid mouthguard biosensor with integrated wireless electronics. Biosensors and Bioelectronics, v. 74, p. 1061–1068, dez. 2015. Disponível em: <https://linkinghub.elsevier.com/retrieve/pii/S0956566315302876>.
LAHCEN, A. A.; RAUF, S.; BEDUK, T.; DURMUS, C.; ALJEDAIBI, A.; TIMUR, S.; ALSHAREEF, H. N.; AMINE, A.; WOLFBEIS, O. S.; SALAMA, K. N. Electrochemical sensors and biosensors using laser-derived graphene: A comprehensive review. Biosensors and Bioelectronics, v. 168, p. 112565, nov. 2020. Disponível em: <https://linkinghub.elsevier.com/retrieve/pii/S0956566320305571>.
MELO JR., M. A.; SANTOS, L. S. S.; GONÇALVES, M. do C.; NOGUEIRA, A. F. Preparação de nanopartículas de prata e ouro: um método simples para a introdução da nanociência em laboratório de ensino. Química Nova, v. 35, n. 9, p. 1872–1878, 2012. Disponível em: <http://www.scielo.br/scielo.php?script=sci_arttext&pid=S0100-40422012000900030&lng=pt&nrm=iso&tlng=en>.
RAUF, S.; LAHCEN, A. A.; ALJEDAIBI, A.; BEDUK, T.; ILTON DE OLIVEIRA FILHO, J.; SALAMA, K. N. Gold nanostructured laser-scribed graphene: A new electrochemical biosensing platform for potential point-of-care testing of disease biomarkers. Biosensors and Bioelectronics, v. 180, p. 113116, maio 2021. Disponível em: <https://linkinghub.elsevier.com/retrieve/pii/S0956566321001536>.
SAMOSON, K.; SOLEH, A.; SAISAHAS, K.; PROMSUWAN, K.; SAICHANAPAN, J.; KANATHARANA, P.; THAVARUNGKUL, P.; CHANG, K. H.; LIM ABDULLAH, A. F.; TAYAYUTH, K.; LIMBUT, W. Facile fabrication of a flexible laser induced gold nanoparticle/chitosan/ porous graphene electrode for uric acid detection. Talanta, v. 243, p. 123319, jun. 2022. Disponível em: <https://linkinghub.elsevier.com/retrieve/pii/S0039914022001151>.
TOLENTINO, E. de S. Avaliação do pH da saliva e da saburra lingual antes e após a utilização de soluções enxaguantes orais e sua relação com parâmetros de halitose. 2009. Universidade de São Paulo, Bauru, 2009. Disponível em: <http://www.teses.usp.br/teses/disponiveis/25/25132/tde-28052009-145508/>.
XU, G.; JARJES, Z. A.; DESPREZ, V.; KILMARTIN, P. A.; TRAVAS-SEJDIC, J. Sensitive, selective, disposable electrochemical dopamine sensor based on PEDOT-modified laser scribed graphene. Biosensors and Bioelectronics, v. 107, p. 184–191, jun. 2018. Disponível em: <https://linkinghub.elsevier.com/retrieve/pii/S095656631830126X>.
XU, P.; LI, R.; TU, Y.; YAN, J. A gold nanocluster-based sensor for sensitive uric acid detection. Talanta, v. 144, p. 704–709, nov. 2015. Disponível em: <https://linkinghub.elsevier.com/retrieve/pii/S0039914015301533>.