Autores
Gamboa Suárez, M.A. (UNIVERSIDAD INDUSTRIAL DE SANTANDER) ; Combariza, M.Y. (UNIVERSIDAD INDUSTRIAL DE SANTANDER) ; Blanco Tirado, C. (UNIVERSIDAD INDUSTRIAL DE SANTANDER)
Resumo
Bacterial cellulose (BC) is a biodegradable polymer that can be synthesized from
agro-industrial waste. However, its hydrophilic nature limits its application in
areas such as food packaging, where moisture control is essential. In this
contribution, we test the effect of BC surface modification on the production of
a hydrophobic material. The formation of amides, from amines coupled to
carboxylic units of oxidized cellulose BC-TEMPO (BC-TOCN), allows modulating its
hydrophilic properties.
Characterization by IR, TGA, SEM and DLS suggests an effective modification of
its surface. Contact angle (CA) and surface free energy (SFE) analysis indicate
changes in hydrophobicity. Amidation increases the contact angle from 29° for
BC-TOCN to 80° with BC-TOCN-AMD C-12 and 147° with BC-TOCN-AMD C-18.
Palavras chaves
Bacterial cellulose ; amidated cellulose ; hydrophobization
Introdução
Plastic waste's pollution of surface waters is rising at the planetary level.
Synthetic plastics are recalcitrant to microbial degradation and generate CO2
emissions causing negative impacts on ecosystems (COSTELLO, et al., 2009; SHEN,
et al., 2020). Biodegradable polymers (PBs) emerge as a solution to pollution by
synthetic plastic waste. The production of PBs by biotechnological pathways
using inexpensive carbon sources, especially by-products of agro-industrial
processes, is a very active research area. Colombia is a country with an
agricultural vocation with abundant residual biomass. Cacao beans production
generates by-products such as cocoa mucilage exudate (CME), a liquid with high
nutrient content, valuable as a carbon source for culture media in
biotechnological processes. We previously demonstrated the use of CME for
bacterial cellulose (BC) production.
Structurally similar to plant cellulose, BC is a linear homopolymer formed by
condensation of glucose molecules. BC is produced, by static or dynamic
fermentations, by a group of microorganisms, among which the Gluconacetobacter
xilynus stands out (SAAVEDRA SANABRIA, et al., 2021).
BC exhibits good mechanical properties, high crystallinity, non-toxicity,
biocompatibility, and biodegradability. BC finds uses in many areas, such as
cosmetics, pharmaceuticals, biomaterials, and medicine (WANG, TAVAKOLI, & TANG,
2019). However, BC's hydrophilic nature restricts its use in specific
applications such as food packaging, particularly for products susceptible to
moisture damage. Thus, BC hydrophobization can modulate its hydrophobic
character and improve its properties as an additive in biodegradable flim
packaging materials (BALASUBRAMANIAM, PATEL, & NAYAK, 2020).
Currently, several techniques are documented to improve BC´s moisture barrier
capacity, such as esterification with organic acids (PENG, CHANG, KUMAR, MOON, &
YOUNGBLOOD, 2016), amination (SIRVIO, VISANKO, LAITINEN, AMMALA, & LIIMATAINEN,
2016) and surface amidation (TAUBNER, COPÍKOVÁ, HAVELKA , & SYNYTSYA, 2013). We
previously reported a surface amidation strategy to modify commercial
microcrystalline cellulose, and Fique fibers cellulose using TBTU uronium salt
[O-(1H benzotriazol-1-yl)-N, N,N',N'-tetramethyluronium tetrafluoroborate] as
coupling agent, TEMPO-oxidized nanocellulose (BC-TOCN), and a primary amine
(GÓMEZ, COMBARIZA, & BLANCO TIRADO, 2017). The modified material exhibits
increased contact angle values, up to five times higher than the CA for the
unmodified BC. This material displays favorable properties such as
biodegradability and hydrophobicity, making it a potential additive for food
packaging. On the other hand, BC is produced using CME, a residual by-product
from the cocoa bean processing, thus avoiding the negative environmental impacts
associated with discarding effluents with a high organic load into soils and
surface water.
Material e métodos
• Bacterial cellulose
The BC was biosynthesized by Gluconacetobacter xylinus in reactors with an
operating volume of 3 L containing a supplemented medium and CME as a carbon
source. BC is produced extracellularly; the film is washed and sterilized once
the process finishes.
• Bacterial cellulose TEMPO oxidation
TEMPO ( 0.016 g) and NaBr (0.1 g) were added to a 1% (w/v) BC aqueous
suspension. NaClO was added to the mixture dropwise, maintaining a molar ratio
of 1:6 cellulose: NaClO. The reaction flask was placed in an ultrasonic bath for
four hours while keeping the pH at 10.5 by NaOH addition. pH stabilization
indicates reaction completion; at this point, ethanol is added to stop the
catalytic reaction cycle. The reaction mixture was centrifuged, and the solid
was washed with water and HCl (0.1 M) until neutral pH. Finally, an ultrasonic
probe was used to obtain a uniform dispersion of BC-TOCN.
• BC-TOCN amidation
TBTU dissolved in dimethylformamide (DMF) was added to a BC-TOCN solution in a
2:1 TBTU:BC-TOCN molar ratio. The reaction mixture was stirred at room
temperature for 30 minutes, keeping the pH basic. A primary amine solution
(dodecylamine or octadecylamine) in DMF (molar ratio 4:1 amine group:COOH units
in BC-TOCN), was added under constant stirring at room temperature for 2 hours.
The solution was then filtered and washed with methanol, HCl, and water to
eliminate unreacted amines. Finally, frozen and lyophilized materials were
labeled BC-TOCN AMD C-12 and BC-TOCN AMD C-18.
• Characterization
The degree of oxidation and the colloidal stability of the BC-TOCN were
evaluated by means of conductometric titration curves and ζ potential
measurements, respectively. Thermal stability was also tested by
thermogravimetry (TGA). The morphological changes and dimensions of the modified
cellulose surface were observed by scanning electron microscopy (SEM). Likewise,
its structure was studied by means of infrared spectroscopy (FT-IR) and X-ray
diffraction (XRD). Additionally, the hydrophobicity of the materials was
evaluated with contact angle measurements.
Resultado e discussão
Bacterial cellulose TEMPO oxidized nanofibers (BC-TONC) exhibit a degree of
oxidation (OD) of 0.26 and 1.53 mmol of COOH/g of cellulose. These
characteristics indicate a reactive surface loaded with carboxylic groups,
suitable for amide bonds formation. Figure 1 shows the IR spectrum of all the
synthesized materials, together with a table showing the most characteristic
bands. For BC, the broad band at 3341 cm-1 corresponds to stretching vibrations
of the O-H bond that is present in the cellulose structure. The small signal
seen at 2882 cm-1 is characteristic of stretching of the C-H bond (OH, et al.,
2005). Additionally, the band at 1630 cm-1 is due to the bending of the H-O-H
bond of water, given BC-TOCN´s highly hydrophilic nature. The band at 1316 cm-1
corresponds to the (C6) CH2 stretching. Additionally, at 1159 and 1107 cm-1
signs of stretching and symmetric and asymmetric elongations of the C-O-C were
observed. The signals at 1056 and 1031 cm-1 are characteristic of skeletal
vibrations involving C-O stretching of the pyranose ring (KONDO & SAWATARI,
1996; DUBEY, et al., 2017). On the other hand, for the BC-TOCN, the band at 1601
cm-1 corresponding to the COO-stretching is observed, which confirms the
presence of carboxylate moieties in BC-TOCN. Additionally, in this band a
shoulder is observed at 1632 cm-1, which is due to the presence of aldehyde and
carbonyl groups, which end up overlapping (CHITBANYONG, PISUTPICHED,
KHANTAYANUWONG, THEERAGOOL, & PUANGSIN, 2020). Additionally, in BC-TOCN-AMD-C12
and C18, as a product of the amidation of the material, a high increase in the
signals attributed to the stretching of the methyl (–CH3) and methylene (–CH2)
groups is observed at 2954 y 2850 cm-1 of the aliphatic chains of the amines.
And significantly, the signals that confirm the presence of the amine group
correspond to the overlapping broad band of amide I and amide II at 1643 y 1569
cm-1 respectively (LASSEUGUETTE, 2008; GÓMEZ, COMBARIZA, & BLANCO TIRADO, 2017).
Likewise, Figure 1 shows the SEM images of these materials. In the BC (Figure
1.a) the morphology of the bacterias that produce bacterial cellulose, the
gluconacetobacter xylinus, can be observed, even adhered to it since its
production occurs extracellularly (SAAVEDRA SANABRIA, et al., 2021). In
addition, when comparing the change that occurred after oxidation via TEMPO and
mechanical defibrillation (Figure 1.b), the separated BC-TOCN can be observed
forming networks, with diameters at the nanometric scale. Researchers report
similar morphologies and sizes, such as those reported by OVALLE SERRANO, GÓMEZ,
BLANCO TIRADO, & COMBARIZA (2018) who observed diameters around 100 nm, and
those reported by LIU, et al., (2016) with diameters between 200 nm and 1.5 μm.
Additionally, for BC-TOCN-AMD-C12 and C18 (Figure 1.c.d) the cellulose fibers
are observed, some wider than others and adhered to them, which could correspond
to the coupling of the grafted amines in the form of sheets. However, it can be
inferred that its morphology does not change drastically as reported in similar
studies GÓMEZ, COMBARIZA, & BLANCO TIRADO (2017).
On the other hand, regarding thermal degradation processes, for BC the point of
maximum decomposition of its structure occurs at 342 °C with a mass loss of
58.99% and for BC-TOCN it occurs at 303 °C , that is, there is a decrease in
thermal stability (FUKUZUMI, SAITO, OKITA, & ISOGAI, 2010) with a mass loss of
19.71%. This is explained by the presence of carboxylic units that favor the
decomposition of BC, facilitating the transitions from solid phase to gas
through decarboxylation reactions. Also for the BC-TOCN, a shoulder can be seen
at a temperature of 232 °C with a weight loss of 14.44%, which corresponds to
the decomposition of the carboxylic units on the BC-TOCN surface. Additionally,
in BC-TOCN-AMD-C12 and C18 thermal decomposition events were observed near 200
°C, possibly attributed to the volatilization of the hydrocarbon chains of the
primary amines (CALDERÓN VERGARA, OVALLE SERRANO, BLANCO TIRADO, & COMBARIZA,
2019). In addition, the thermal degradation temperature for the amidated
derivatives at 318 °C for AMD C-12 BC-TOCN and 317 °C for AMD C-18 BC-TOCN, is
higher than for BC-TOCN. This indicates that the coupling of the primary amines
leads to an apparent improvement in the thermal resistance of BC-TOCN.
Additionally, the colloidal stability of BC-TOCN was analyzed by means of the Z
potential. Thus, a value of -60.8 mV was obtained, inferring a good colloidal
stability probably in response to the amount of negatively charged carboxyl
groups COO- on the surface of the cellulose structure, which causes
electrostatic repulsions that prevent the suspension from agglomerating
(MAHENDRAN & RANJITHA KUMARI, 2016).
On the other hand, the contact angle (CA) tests were performed with a double
injector using the double sessile drop measurement method, depositing drops of
water and diiodomethane, as shown in figure 2. For the BC-TOCN films, a contact
angle to water of 29°, evidencing its highly hydrophilic nature corresponding to
the presence of polar groups (–OH and –COOH) on the surface. As for the
materials modified with primary amines, an increase from 29° to 80° was observed
with BC-TOCN-AMD-C-12 and 147° with BC-TOCN-AMD-C-18, corresponding to the
presence of the alkyl chains of dodecylamine and octadecylamine nonpolar nature,
which coupled to the BC-TOCN surface. Comparing the results obtained, it is
observed that the increase in hydrophobicity obtained with octadecylamine is
higher than in other studies. However, with dodecylamine this increase was not
as significant, but higher than that reported by GÓMEZ, COMBARIZA, & BLANCO
TIRADO (2017). In addition, with the experimental values of the contact angles
of water and diiodomethane with the films, the surface free energy (SFE) was
obtained γ_s and the polar components γ_s^p and dispersive γ_s^d (Figure 2).
Indeed, it is observed that BC-TOCN obtained values of SFE and its components
very similar to those obtained by the investigation of CALDERÓN VERGARA, OVALLE
SERRANO, BLANCO TIRADO, & COMBARIZA (2019) with BC-TOCN from fique.
Additionally, they are within the ranges reported by authors such as KHOSHKAVA &
KAMAL (2013) in nanocrystalline cellulose with SFE values of 66 mJ/m2
characteristic of materials with a hydrophilic nature. Likewise, as the higher
value of the polar component on the dispersive that indicates hydrogen bond,
inductive and acid-base interactions. Unlike the materials modified with amines,
with which it was possible to demonstrate that amidation decreases the SFE of
the materials, especially the one coupled with octadecylamine whose value was
7,5 mJ/m2, very similar to that reported by CALDERÓN VERGARA, OVALLE SERRANO,
BLANCO TIRADO, & COMBARIZA (2019). Additionally, the polar component decreases
drastically, being lower than the dispersive component, which indicates Van der
Waals type interactions, characteristic of hydrophobic materials.
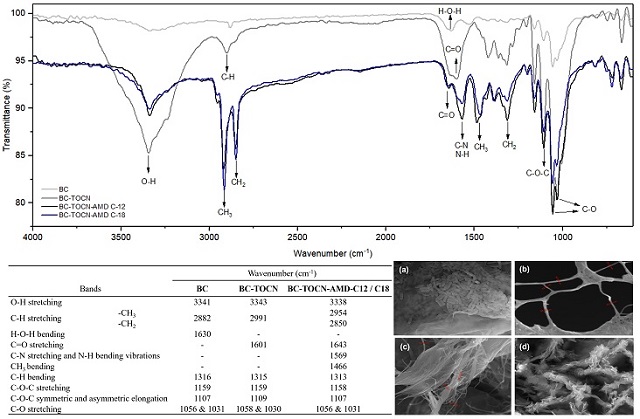
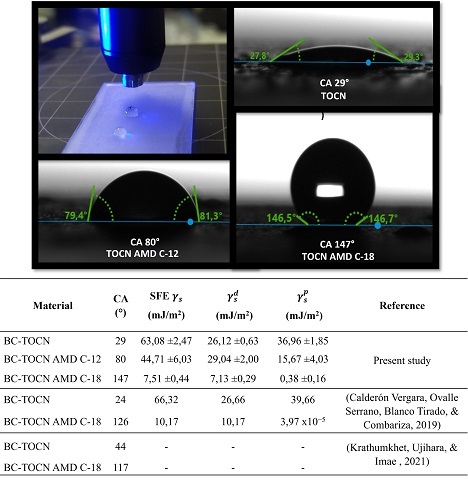
Conclusões
Contact angle measurement indicated the coupling of the primary amines with the
procedures used. This is supported by the appearance of new signals in the IR
spectrum attributed to the formation of amide bonds. Likewise, a slight increase
in the decomposition temperature of the amidated materials with respect to TOCN
was observed. On the other hand, morphological and dimensional changes of the BC
surface and its modifications were observed, inferring that the oxidation via
TEMPO combined with ultrasound effectively leads to the separation of the
nanofibers. In addition, the apparent coupling of the amines to TOCN is observed.
Likewise, as a result of CA and SFE, the hydrophobization of the material was
corroborated, reaching contact angles with octadecylamine of up to 147° and SFE
values of 7.5 mJ/m2, which are consistent with its chemical structure. Which
indicates that the resulting materials have good potential to be used as additives
in biopackaging given their biocompatibility, degradability, along with
hydrophobicity and improvement in their properties.
Agradecimentos
We thank the Guatiguará Technology Park at Universidad Industrial de Santander for
infrastructural support. To Minciencias and the Universidad Industrial de
Santander for financing the postgraduate project of Maria Andrea Gamboa.
Referências
BALASUBRAMANIAM, S. L., PATEL, A. S., & NAYAK, B. (2020). Surface modification of cellulose nanofiber film with fatty acids for developing renewable hydrophobic food packaging. Food Packaging and Shelf Life, 26. doi:10.1016/j.fpsl.2020.100587
CALDERÓN VERGARA, L. A., OVALLE SERRANO, S. A., BLANCO TIRADO, C., & COMBARIZA, M. Y. (November de 2019). Influence of post-oxidation reactions on the physicochemical properties of TEMPO-oxidized cellulose nanofibers before and after amidation. Cellulose, 27, 1273–1288. doi:10.1007/s10570-019-02849-4
CHITBANYONG, K., PISUTPICHED, S., KHANTAYANUWONG, S., THEERAGOOL, G., & PUANGSIN, B. (November de 2020). TEMPO-oxidized cellulose nanofibril film from nano-structured bacterial cellulose derived from the recently developed thermotolerant Komagataeibacter xylinus C30 and Komagataeibacter oboediens R37–9 strains. International Journal of Biological Macromolecules, 163(15), 1908-1914. doi:10.1016/j.ijbiomac.2020.09.124
COSTELLO, A., ABBAS, M., ALLEN, A., BALL, S., BELLAMY, R., FRIEL, S., . . . JOHNSON ANNE. (2009). Managing the health effects of climate change. Lancet, 373(9676), 1693-1733.
DUBEY, S., SHARMA, R. K., AGARWAL, P., SINGH, J., SINHA, N., & SINGH, R. P. (March de 2017). From rotten grapes to industrial exploitation: Komagataeibacter europaeus SGP37, a micro-factory for macroscale production of bacterial nanocellulose. International Journal of Biological Macromolecules, 96, 52-60. doi:10.1016/j.ijbiomac.2016.12.016
FUKUZUMI, H., SAITO, T., OKITA, Y., & ISOGAI, A. (September de 2010). Thermal stabilization of TEMPO-oxidized cellulose. Polymer Degradation and Stability, 95(9), 1502-1508. doi:10.1016/j.polymdegradstab.2010.06.015
GÓMEZ, F. N., COMBARIZA, M. Y., & BLANCO TIRADO, C. (2017). Facile cellulose nanofibrils amidation using a ‘one-pot’. Cellulose, 24, 717–730. doi:10.1007/s10570-016-1174-9
KONDO, T., & SAWATARI, C. (1996). A Fourier transform infrared spectroscopic analysis of the character of hydrogen bonds in amorphous cellulose. Polymer, 37(3), 393-399.
MAHENDRAN, G., & RANJITHA KUMARI, B. D. (December de 2016). Biological activities of silver nanoparticles from Nothapodytes nimmoniana (Graham) Mabb. fruit extracts. Food Science and Human Wellness, 5(4), 207-218. doi:10.1016/j.fshw.2016.10.001
OH, S. Y., YOO, D. I., SHIN, Y., KIM, H. C., KIM, H. Y., CHUNG, Y. S., . . . YOUK, J. H. (September de 2005). Crystalline structure analysis of cellulose treated with sodium hydroxide and carbon dioxide by means of X-ray diffraction and FTIR spectroscopy. Carbohydrate Research, 340, 2376–2391. doi:10.1016/j.carres.2005.08.007
OVALLE SERRANO, S. A., GÓMEZ, F. N., BLANCO TIRADO, C., & COMBARIZA, M. Y. (February de 2018). Isolation and characterization of cellulose nanofibrils from Colombian Fique decortication by-products. Carbohydrate Polymers, 189, 169-177. doi:10.1016/j.carbpol.2018.02.031
PENG, S. X., CHANG, H., KUMAR, S., MOON, R. J., & YOUNGBLOOD, J. P. (march de 2016). A comparative guide to controlled hydrophobization of cellulose nanocrystals via surface esterification. Cellulose, 23(3), 1825-1846.
SAAVEDRA SANABRIA, O. L., DURÁN, D., CABEZAS, J., HERNÁNDEZ, I., BLANCO TIRADO, C., & COMBARIZA, M. Y. (November de 2021). Cellulose biosynthesis using simple sugars available in residual cacao mucilage exudate. Carbohydrate Polymers, 274(15). doi:10.1016/j.carbpol.2021.118645
SHEN, M., SONG, B., ZENG, G., ZHANG, Y., HUANG, W., WEN, X., & TANG, W. (August de 2020). Are biodegradable plastics a promising solution to solve the global plastic pollution? Environmental Pollution, 263.
SIRVIO, J. A., VISANKO, M., LAITINEN, O., AMMALA, A., & LIIMATAINEN, H. (2016). Amino-modified cellulose nanocrystals with adjustable hydrophobicity from combined regioselective oxidation and reductive amination. Carbohydrate Polymers, 136, 581-587. doi:10.1016/j.carbpol.2015.09.089
TAUBNER, T., COPÍKOVÁ, J., HAVELKA , P., & SYNYTSYA, A. (May de 2013). Preparation of amidated derivatives of monocarboxy cellulose. cellulose, 20, 2045–2055. doi:10.1007/s10570-013-9938-y
WANG, J., TAVAKOLI, J., & TANG, Y. (September de 2019). Bacterial cellulose production, properties and applications with different culture methods – A review. Carbohydrate Polymers, 219, 63-76. doi:10.1016/j.carbpol.2019.05.008